By Ross S. Stein, Ph.D., Temblor; Chris Rollins, Ph.D., Michigan State University; Volkan Sevilgen, M.Sc., Temblor; and Tiegan Hobbs, Postdoctoral Seismic Risk Scientist, Temblor (@THobbsGeo)
The 50-km-long rupture has triggered widely dispersed aftershocks. However, almost none of these struck on the faults that the M 7.1 brought closer to failure: The major Garlock Fault; or the Blackwater, Panamint Valley, or Sierra Nevada Faults.
Citation: Ross S. Stein, Chris Rollins, Volkan Sevilgen, and Tiegan Hobbs, (2019), M 7.1 SoCal earthquake triggers aftershocks up to 100 mi away: What’s next?, Temblor, http://doi.org/10.32858/temblor.038
A fault out of nowhere
It is now clear that the Ridgecrest M 7.1 earthquake struck on a straight, rather simple, right-lateral (see half-arrows in the map below) rupture. That is ironic, since nothing at the ground surface had given this fault away before it fired off the quake. Some of the world’s best field geologists had scoured this area for the past 50 years, because faulting is much more readily mapped in this arid, undeveloped landscape, and because of its association with geothermal activity. The latter, geothermal activity, has heated, stretched, and locally weakened the crust here. Further, this region has had a high rate of moderate shocks since seismic recording technology was enhanced in about 1980; they gave no hint of such a continuous fault.
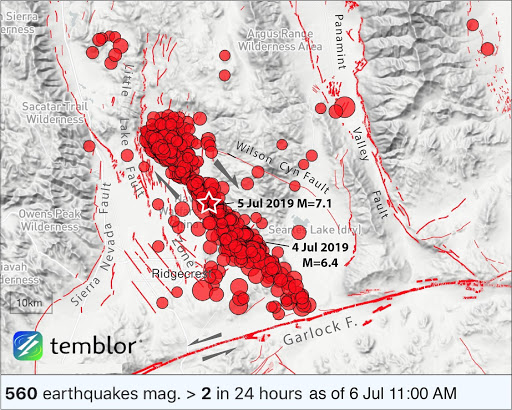
Chicken-or-egg riddle answered?
In a chicken-or-egg conundrum, it’s never been clear whether this region is the site of continuing off-fault aftershocks of the 1872 M~7.6 Owens Valley earthquake, or whether, because the crust is heated, weak, and shocks are abundant, all of the local stress is continuously being released. If that were the case, then the 1872 shock may have been arrested just to the north because it ran out of stress (Stein, 2016).
Now, the Ridgecrest M 7.1 has delivered the answer: There was plenty of accumulated stress, enough to permit a quake with 3 m (10 ft) of slip. That suggests that one can, indeed, have aftershocks 150 years after very large mainshocks, an argument we advanced in Toda and Stein (2018). This was inspired by the work of John Adams (Natural Resources Canada), John Ebel (Boston College) and Seth Stein (Northwestern University).
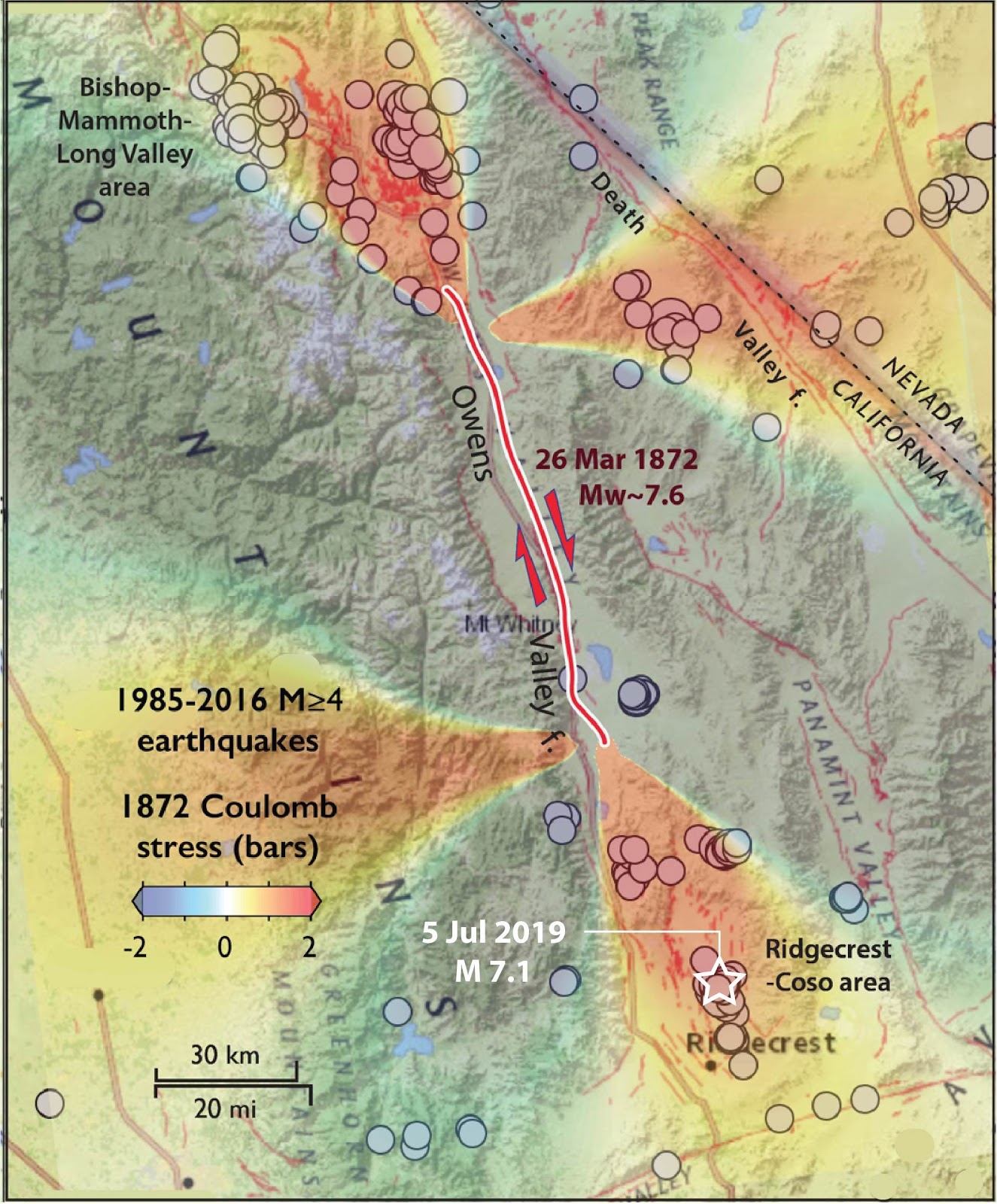
Yet another shoe to drop?
To assess which faults have now been brought closer to failure by the M=6.4 and M=7.1 earthquakes, we calculated the Coulomb stress transfer from them to nearby faults, below. The hypothesis is that earthquakes interact by the transfer of stress: they are not isolated events, but always in a kind of ‘conversation’ moderated by stress. Slip on faults is promoted when the faults are sheared in the same way that they naturally slip in earthquakes, and when they faults are unclamped (two sides pulled apart, making slip easier); those sites are colored red below. Faults are inhibited from failure when the imparted shear stress is opposite to the way in which they slip in earthquakes, and when the faults are clamped; those ‘stress shadows’ are colored blue.
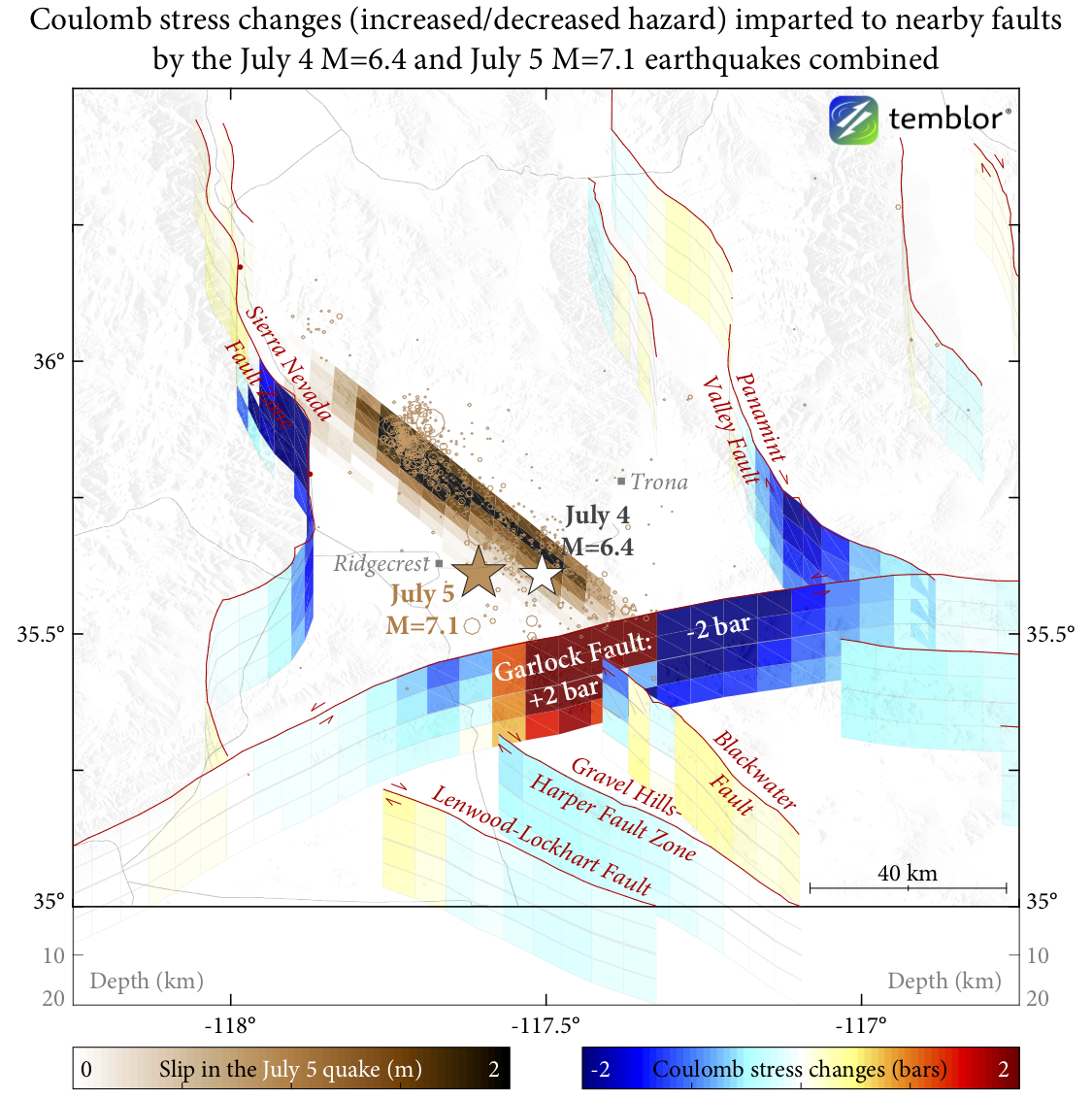
The biggest loser is a 30-km stretch of the Garlock Fault just south of the action that was hit by a Coulomb stress increase in excess of 2 bars. Remarkably, this section has not lit up in aftershocks. Could this stretch of the Garlock be waiting to go all in one big event? That could be, but note that Coulomb stress has been decreased on either side of the red zone, nominally making failure less likely on those sections. There might be another explanation for the silence of the red zone: that the Garlock has been rotated so far out of alignment with the tectonic stress direction that it can only slip if it is very slippery. So, despite its length (280 km, 170 mi) and the geological offsets along it, it is possible that the Garlock isn’t as much of a major tectonic player these days. There have been prehistoric earthquakes on it, which would contradict this inference. In any case, the Garlock is an oddity.
Smaller but still significant stress increases are calculated for the Blackwater, Panamint Valley, and Sierra Nevada Faults. There have been a few aftershocks near these faults, so they should all be watched.
Dynamically triggered aftershocks extend to Nevada
An unmistakable jet of aftershocks can be seen extending about 150 km (90 mi) to the northeast of the mainshock. These could be ‘busted locations,’ when the seismic network is overwhelmed by the waves from multiple quakes and therefore unable to accurately locate events. Accordingly, this observation is preliminary. But it looks remarkably like the remote aftershocks of the 1992 M 7.3 Landers earthquake, also in the Eastern California Shear Zone, discovered by Hill et al. (1993), and seen many times since for many, but certainly not all—other large quakes.
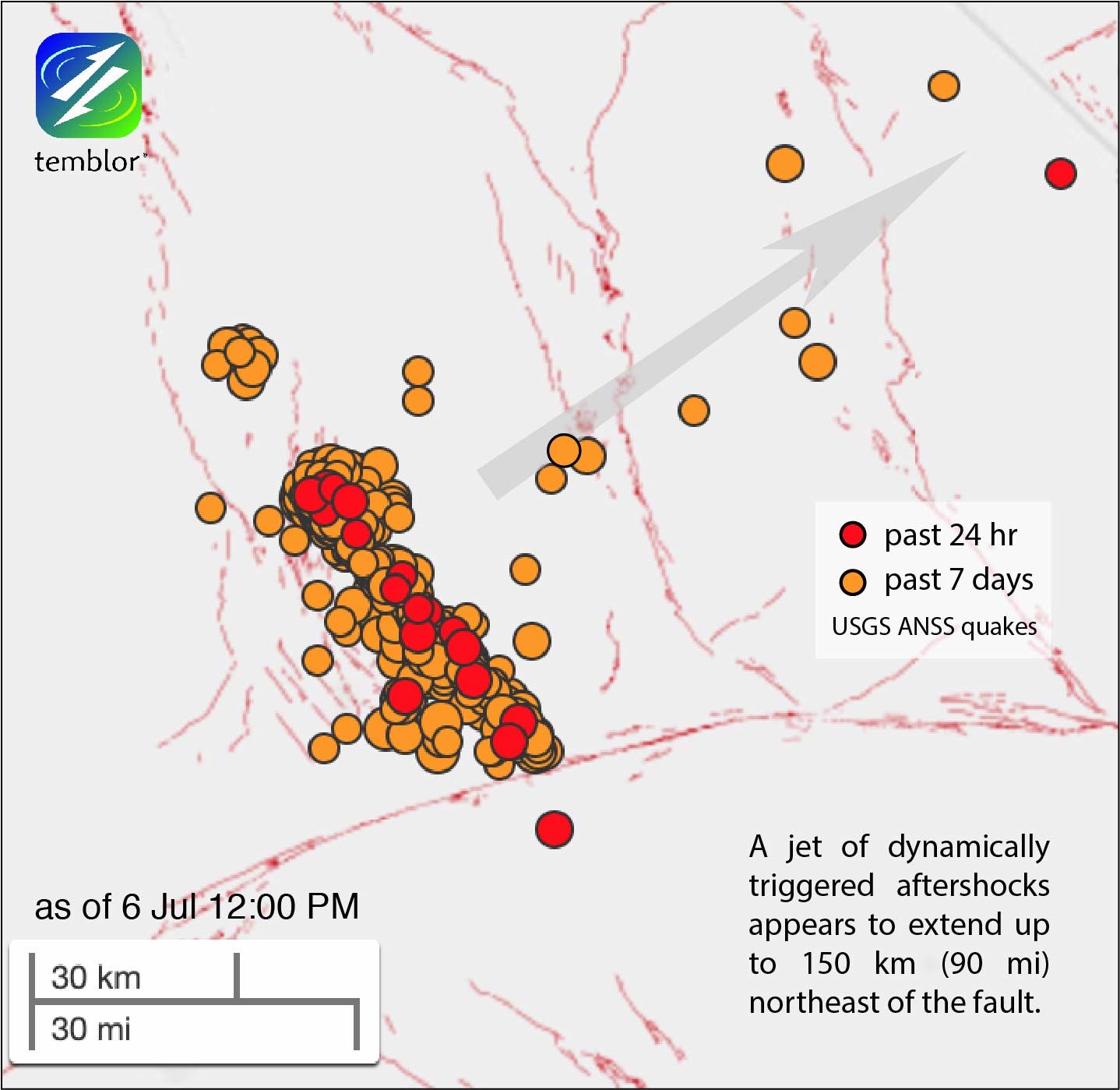
Comparing the 24 hr before the mainshock and the elapsed 17 hr afterwards (below) all but eliminates the possibility that these events are simply ‘background’ shocks that occur all the time. Rather, the remote shocks must be the product of the mainshock if they are indeed real quakes. But they cannot be explained by the permanent Coulomb stress changes that we previously calculated, because those stresses diminish nearly to zero about 75 km away in this case. Instead, these stresses are carried by the seismic waves, and so are transient. Somehow, they stimulate faults to slip after a delay of some hours.
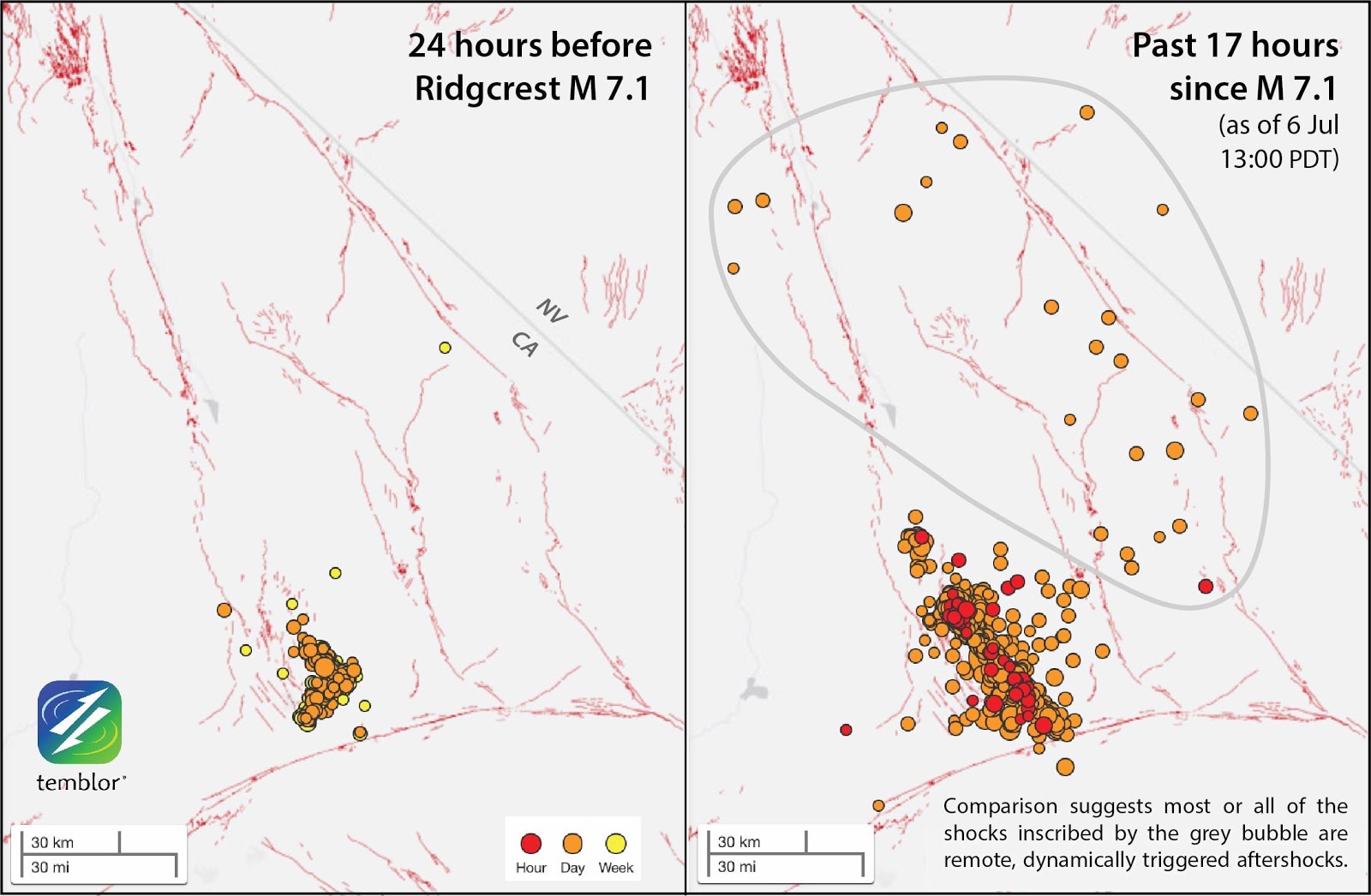
Another mystery
We would expect the dynamic stresses carried by the seismic waves to be strongest in the direction that the fault unzipped—toward the northwest and southeast—but instead the remote shocks we see are widely distributed to the northeast. Once again, a quake has broken all our rules.
Parting shot
After a M 4.0 was followed 30 min later by a M 6.4, and the M 6.4 was followed 34 hr later by a M 7.1, we should all ask ourselves if we are ready for whatever this earthquake sequence—or the next one—may bring. That means putting a whistle on your keychain. It means having an earthquake emergency kit in your home, car, and place of work (http://temblor.net/earthquake-insights/earthquake-emergency-kit-for-a-gift-220/). It means having a communication plan, and a solar charger and cell phone battery in your kit. It means making sure your home and its contents are as seismically resilient as possible, and asking whether you could deal with the repair cost and dislocation if it is not. Empower yourself in the face of a trembling earth.
References
Hauksson, E., & Unruh, J. (2007). Regional tectonics of the Coso geothermal area along the intracontinental plate boundary in central eastern California: Three‐dimensional Vp and Vp/Vs models, spatial‐temporal seismicity patterns, and seismogenic deformation. Journal of Geophysical Research, 112(B6).
Hill, David P, P. A. Reasenberg, A. Michael, W. J. Arabaz, G. Beroza, D. Brumbaugh, J. N. Brune, R. Castro, S. Davis, D. dePolo, W. L. Ellsworth, J. Gomberg, S. Harmsen, L. House, S. M. Jackson, M. J. S. Johnston, L. Jones, R. Keller, S. Malone, L. Munguia, S. Nava, J. C. Pechmann, A. Sanford, R. W. Simpson, R. B. Smith, M. Stark, M. Stickney, A. Vidal, S. Walter, V. Wong, J. Zollweg (1993), Seismicity Remotely Triggered by the Magnitude 7.3 Landers, California, Earthquake, Science, 260, 1617-1623, DOI: 10.1126/science.260.5114.1617
McAuliffe, L. J., J. F. Dolan, E. Kirby, C. Rollins, B. Haravitch, S. Alm, and T. M. Rittenour (2013), Paleoseismology of the southern Panamint Valley fault: Implications for regional earthquake occurrence and seismic hazard in southern California, J. Geophys. Res., 118, 5126–5146, doi:10.1002/jgrb.50359.
Stein, Ross S. (2016), Eastern California’s intense seismic and geothermal activity: Chicken or Egg? Temblor, http://temblor.net/earthquake-insights/eastern-california-intense-seismic-and-geothermal-activity-594/
Shinji Toda and Ross S. Stein (2018), Why Aftershock Duration Matters for Probabilistic Seismic Hazard Assessment, Bull. Seismol. Soc. Amer., 108, 1414-1426, https://doi.org/10.1785/0120170270
- Earthquake science illuminates landslide behavior - June 13, 2025
- Destruction and Transformation: Lessons learned from the 2015 Gorkha, Nepal, earthquake - April 25, 2025
- Knock, knock, knocking on your door – the Julian earthquake in southern California issues reminder to be prepared - April 24, 2025